Laser-plasma accelerators (LPA) propel high-energy particles over short distances thanks to intense, ultrashort pulses of laser light. These accelerators can provide high-quality particle beams (of electrons, protons, and X‑ray photons) for radio-biological studies that will help scientists better understand how radiation damages DNA and, ultimately, optimise cancer treatments that make use of ionising radiation.
Recent studies have shown that the biological effect of radiation depends not only on the total dose delivered, but also the time and the rate over which it is delivered. At the LOA (Laboratory of Applied Optics), we are studying the effect of ultra-high dose rates in order to develop irradiation protocols capable of increasing the differential response between healthy and cancerous cells.
The lasers we use produce very short pulses, in the picosecond (10-12 s) or femtosecond (10-15 s) range and are very intense – so much so that when a laser pulse strikes the target material, it immediately ionises it, transforming it into a plasma, a state of matter in which electrons and ions form a kind of “soup” with a collective motion. Thus, detached from their nuclei, the electrons react to the laser field through the plasma.
In the case of a very dense plasma, in which the laser fails to propagate, the electrons escaping from the target cause it to explode, thus accelerating the ions in it. The result: ions in the mega-electronvolts (MeV) energy range are produced over lengths as short as millimetres.
In the case of a low-density plasma, the propagation of the laser pulse produces a wake wave behind it that propagates at the speed of light. The electrons trapped in this wave are accelerated – like a surfer on a wave – and thus gain energy. Electric field gradients as high as those produced by this strategy (around (100 GeVm-1) are not possible with conventional structures (radio frequency cavities), which can reach about just 0.1 GeVm-1.
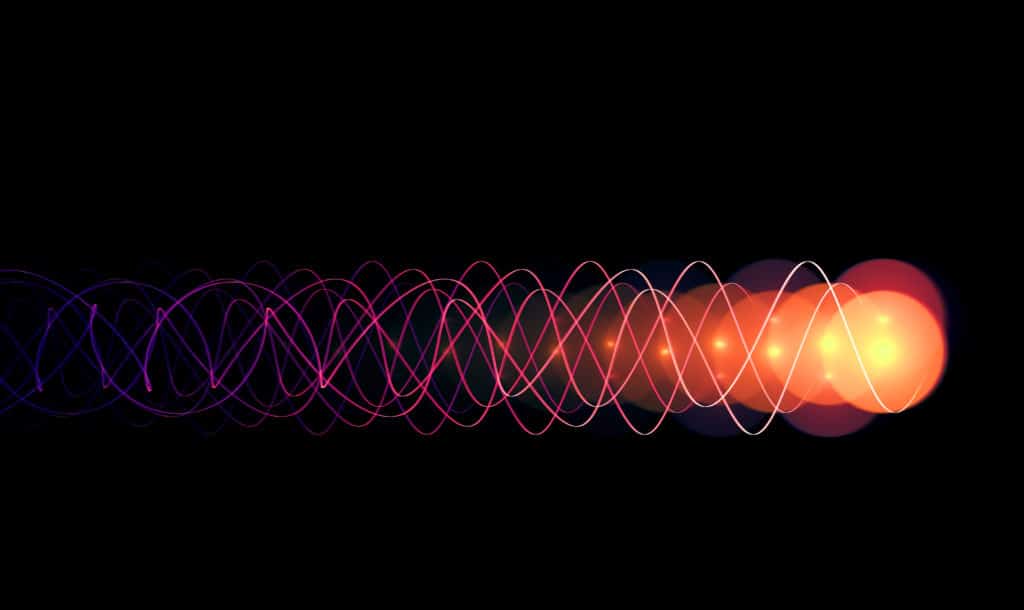
Ionising radiation is used in medicine for many purposes: from imaging to diagnosis and cancer treatment. There are a range of possible therapies based on the harmful effect of this radiation on living organisms. Electron beams damage cancer cells in a very similar way to high-energy photons, which are the most common radiotherapy modality in clinical practice. This is because high-energy electrons convert much of their kinetic energy into X‑ray photons by “bremsstrahlung”, which triggers a cascade of electrons, positrons, and photons, and also ionises the material by inelastic collisions. It is the latter that eventually damages cancer cells, either by directly ionising the DNA or by indirectly creating radicals that damage it.
Differences in radioresistance between healthy and cancer cells
In any type of therapy, there is a differential between the desired effect and the unintended side effects. In radiotherapy, the toxicity of ionising radiation on living organisms is exploited. Basically, healthy cells have a slightly higher radioresistance than cancer cells. This difference allows the development of ionising radiation dose delivery protocols in which the harmful effect on healthy cells is less important than the destructive effect on tumour cells.
For laser-accelerated particles, there are two aspects. The first is related to the quality of the particles used. Currently, the most commonly used particles in radio medicine are photons and protons, but each has very different characteristics of dose delivery in biological tissue. Photons deliver their energy according to a decreasing exponential curve, so that there is a maximum dose at the tissue surface and a minimum at depth. Irradiation protocols for deep tumours must therefore be designed in such a way as not to exceed certain doses at entry and to achieve a therapeutic dose at depth, which is not easy.
Photons are widely used in radiotherapy because they are easy to produce, whereas protons are just beginning to be used because they require much larger machines. For example, a photon radiotherapy machine requires a room of about 20 m2 in surface area, whereas proton therapy, which requires a cyclotron, must be installed in a building several hundred square metres in size.
Electrons, on the other hand, have been little used in the past for several reasons. First, they have a rather flat dose delivery curve. They were also more difficult to accelerate than photons.
The flash effect
This situation changed with the discovery of the flash effect, a phenomenon observed as early as the 1970s and rediscovered at Orsay in the 2000s. Researchers there found that the same therapeutic dose of ionising radiation has a different effect depending on the time scale over which it is delivered.
Researchers found that the same therapeutic dose of ionising radiation has a different effect depending on the time scale over which it is delivered.
The whole field of radiotherapy is based on the postulate that for the same dose, we have an equal response. It’s the same as for a drug, like a dose of paracetamol, for example: the dose determines the biological effect. What we found is that if you deliver the same dose over an extremely short period of time, the therapeutic effect changes. Healthy tissue seems to be much more resistant to the same dose if it is applied over a very short period of time, while the sensitivity of tumour tissue remains unchanged. This means that if this therapeutic dose is delivered over 50 ms rather than 10 minutes, nothing changes for the tumour cells but the damage to healthy tissue is considerably reduced.
The flash effect thus allows for a much more effective and efficient treatment.
The first experiments on the effect (between 2016 and 2020) were carried out with low energy electrons (LEE), which have an energy of less than 5 MeV, simply because these electrons were the most readily available. They can only penetrate to a depth of a few millimetres into the tissue, and therefore cannot be used to treat deep tumours.
Very high energy electrons and fast fractionation
We have now turned our attention to very high energy electrons (VHEE), that is, electrons with energies above 150 MeV, which have been little studied in the past. The beams of these electrons penetrate deep into tissue and can treat tumours that photon irradiation cannot reach. However, a linac-based VHEE treatment system, for example, would have to be compact enough to fit in a hospital treatment room to be competitive with photons.
VHEEs are likely to be more expensive to produce than photons, but cheaper than protons. Depth-dose curves show that in addition to delivering the dose at depth, VHEEs should also be more resilient to unexpected tissue inhomogeneities than X‑rays.
VHEEs can also allow deep tumours to be precisely targeted by concentrating the dose in a small volume. This dose can be controlled, which may be an advantage for the treatment of radiation-resistant tumours. The ability to deliver the dose in a small spot can also benefit ultra-precision therapy, where very small areas of tissue need to be irradiated. In addition, the very low dose at entry and the low distal and proximal doses of VHEE focused beams can help minimise damage to healthy tissue and sensitive organs.
Clinical applications of this type of therapy are still some way off and much work still remains to be done to develop the laser and accelerator technology to make it suitable for the real world. We have called this irradiation modality « fast fractionation » and have started to study its biological effects. Preliminary results show that the rate of laser pulses has an effect on the toxicity of ionising radiation: it is not only the duration of the flash but also the total dose of a single pulse that has an effect on toxicity. We are not able to explain the mechanisms behind the flash effect as yet, but our studies have only just begun. One thing is certain, it holds great promise for the development of highly effective radiotherapy.
Références: